Raptor determines β-cell identity and plasticity independent of hyperglycemia in mice
- PMID: 32439909
- PMCID: PMC7242325
- DOI: 10.1038/s41467-020-15935-0
Raptor determines β-cell identity and plasticity independent of hyperglycemia in mice
Abstract
Compromised β-cell identity is emerging as an important contributor to β-cell failure in diabetes; however, the precise mechanism independent of hyperglycemia is under investigation. We have previously reported that mTORC1/Raptor regulates functional maturation in β-cells. In the present study, we find that diabetic β-cell specific Raptor-deficient mice (βRapKOGFP) show reduced β-cell mass, loss of β-cell identity and acquisition of α-cell features; which are not reversible upon glucose normalization. Deletion of Raptor directly impairs β-cell identity, mitochondrial metabolic coupling and protein synthetic activity, leading to β-cell failure. Moreover, loss of Raptor activates α-cell transcription factor MafB (via modulating C/EBPβ isoform ratio) and several α-cell enriched genes i.e. Etv1 and Tspan12, thus initiates β- to α-cell reprograming. The present findings highlight mTORC1 as a metabolic rheostat for stabilizing β-cell identity and repressing α-cell program at normoglycemic level, which might present therapeutic opportunities for treatment of diabetes.
Conflict of interest statement
The authors declare no competing interests.
Figures
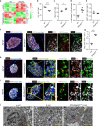
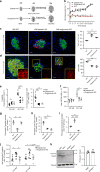
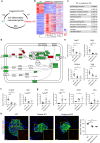
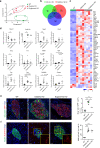
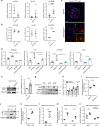
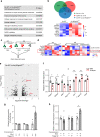
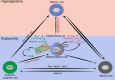
Similar articles
-
Raptor regulates functional maturation of murine beta cells.Nat Commun. 2017 Jun 9;8:15755. doi: 10.1038/ncomms15755. Nat Commun. 2017. PMID: 28598424 Free PMC article.
-
Dual Effect of Raptor on Neonatal β-Cell Proliferation and Identity Maintenance.Diabetes. 2019 Oct;68(10):1950-1964. doi: 10.2337/db19-0166. Epub 2019 Jul 25. Diabetes. 2019. PMID: 31345937
-
Loss of mTORC1 signaling alters pancreatic α cell mass and impairs glucagon secretion.J Clin Invest. 2017 Dec 1;127(12):4379-4393. doi: 10.1172/JCI90004. Epub 2017 Nov 6. J Clin Invest. 2017. PMID: 29106387 Free PMC article.
-
The role of Raptor in lymphocytes differentiation and function.Front Immunol. 2023 May 22;14:1146628. doi: 10.3389/fimmu.2023.1146628. eCollection 2023. Front Immunol. 2023. PMID: 37283744 Free PMC article. Review.
-
Disallowed and Allowed Gene Expression: Two Faces of Mature Islet Beta Cells.Annu Rev Nutr. 2016 Jul 17;36:45-71. doi: 10.1146/annurev-nutr-071715-050808. Epub 2016 May 4. Annu Rev Nutr. 2016. PMID: 27146011 Review.
Cited by
-
Glucose Variability: How Does It Work?Int J Mol Sci. 2021 Jul 21;22(15):7783. doi: 10.3390/ijms22157783. Int J Mol Sci. 2021. PMID: 34360550 Free PMC article. Review.
-
Signaling pathways and intervention for therapy of type 2 diabetes mellitus.MedComm (2020). 2023 Jun 7;4(3):e283. doi: 10.1002/mco2.283. eCollection 2023 Jun. MedComm (2020). 2023. PMID: 37303813 Free PMC article. Review.
-
SORCS2 activity in pancreatic α-cells safeguards insulin granule formation and release from glucose-stressed β-cells.iScience. 2023 Dec 13;27(1):108725. doi: 10.1016/j.isci.2023.108725. eCollection 2024 Jan 19. iScience. 2023. PMID: 38226160 Free PMC article.
-
Susceptibility Loci for Type 2 Diabetes in the Ethnically Endogamous Indian Sindhi Population: A Pooled Blood Genome-Wide Association Study.Genes (Basel). 2022 Jul 22;13(8):1298. doi: 10.3390/genes13081298. Genes (Basel). 2022. PMID: 35893037 Free PMC article.
-
Targeting β-cell dedifferentiation and transdifferentiation: opportunities and challenges.Endocr Connect. 2021 Aug 13;10(8):R213-R228. doi: 10.1530/EC-21-0260. Endocr Connect. 2021. PMID: 34289444 Free PMC article. Review.
References
-
- Weir GC, Bonner-Weir S. Five of stages of evolving β-cell dysfunction during progression to diabetes. Diabetes. 2004;53:S16–S21. - PubMed
Publication types
MeSH terms
Substances
LinkOut - more resources
Full Text Sources
Medical
Molecular Biology Databases
Research Materials